Neurobiology of the Cochlear Nucleus:
Observations Potentially Relevant for the Application of Auditory Brainstem Implants (ABI's) in HumansThere are neurobiological observations that may assist decisions about where to place an auditory brainstem implant in the human brain and how to design it. The data are taken from basic research performed on the brains of various subhuman mammalian species. For the sake of clarity, the species from which results are obtained are not indicated (for identification see references), and it is assumed that the findings listed here are at least qualitatively valid across mammalian brains. The primary sensory neurons of the auditory system, residing in the spiral ganglion, send their central processes into the 8th cranial nerve. The fibers of this sensory nerve root enter the brain stem and terminate on the secondary sensory neurons completely contained in the cochlear nucleus complex (CNC) in a multiple cochleotopic order (1). The CNC consists of subdivisions, which were defined on anatomical (2) or electrophysiological (3) grounds. Although numerous subdivisions can reliably be identified in most mammalian species and a tripartite division is a popular scheme (4), the present purpose justifies to reduce this diversity to two major cochlear nucleus subdivisions: the dorsal cochlear nucleus (DCN) and the three to four times larger ventral cochlear nucleus (VCN). There is a stronger auditory input to VCN as com-pared to DCN. First, whereas all fibers terminate in VCN, almost every fifth auditory nerve fiber falls short of reaching DCN (5). Second, auditory nerve terminals form enormously large and complex synaptic apparatuses, the endbulbs of Held, on cell bodies of VCN neurons, whereas primary auditory terminals in DCN are small and reside mostly on dendrites (6). This difference in input strength is corroborated by physiological observation. Many neurons in VCN have response characteristics to acoustic stimuli very similar to those of the primary sensory neurons and, despite afferents from other sources (7), are almost completely depend upon this input (8). By contrast, neurons in DCN produce more complex response patterns and their spontaneous activity is little affected by loss of cochlear input, indicating a dominance by afferents from sources other than primary auditory axons (8).
Various efferent pathways arise from the CNC subdivisions. These may be classified as belonging to systems mediating binaural hearing ('spatial line') or to systems serving the transmission of sound composition ('spectral line'). Although a clearcut classification of auditory nuclei belonging to either one or the other of these systems is certainly an oversimplification, it appears obvious from connectivity data that functions of the superior olivary complex involve primarily binaural computations, whereas the inferior colliculus is an obligatory way station for the transmission of spectral information to the auditory cortical area, the brain structure ultimately responsible for sound analysis and, in humans, language perception. On this pathway must the auditory brainstem implant research focus. Both VCN and DCN project to the inferior colliculus, but the pathway from VCN is, in terms of the number of participating neurons, about ten times more voluminous (9).
The CNC comprises a considerable number of morphologically distinguishable cell types (10). Some of these pervade the whole CNC (e.g. small cells, granule cells, stellate cells) while others are restricted to VCN (e.g. bushy cells, octopus cells) or DCN (e.g. fusiform cells, cartwheel cells). Each of these types has a specific pattern of inputs and outputs. For instance, primary auditory afferents in the DCN preferentially terminate on the basal dendrites of fusiform cells, whereas the endbulbs of Held are found on VCN neurons, with the largest ones on bushy cells (11). Bushy cells produce primary-like responses to short tone bursts (12) and project preferentially to the superior olive (13). The cochleo-collicular projection ori-ginate from stellate cells in VCN (9,14) responding with a chopper pattern (12), from fusiform cells in DCN showing a pauser-builtup response (15), and from small neurons throughout both subdivisions (9). Most of those neurons in VCN projecting to the inferior colliculus send an axon collateral into DCN (16), supplying this subnucleus with a second major auditory input.
By comparison with secondary neurons of other sensory systems, such as retinal ganglion cells, it is reasonable to expect that each of these neuronal types exerts a specifically patterned action on its target cells. This action may be considered as primarily excitatory or inhibitory, which is determined by the neurotransmitter pre-synaptically released and the types of receptors on the postsynaptic membrane. The primary auditory afferents utilize excitatory amino acids (glutamate and/or aspartate) as transmitter and mainly affect receptors of a non-NMDA type (17). The same substances are likely to be employed by CNC neurons sending their axon outside the cochlear nucleus, in particular those projecting to the inferior colliculus (18). Numerous neurons and terminal swellings in the DCN contain GABA or, even more frequently, glycine (19), both known as potent inhibitory transmitter sub-stances. In VCN, GABAergic cell bodies and terminals are less frequent, but the level of glycinergic cells and fibers as well as the glycine receptor is equally high (20). There also exist neurons in the CNC containing enkephalin, substance P, or other neuropeptides, which are thought to take excitatory or inhibitory action not within milliseconds but within seconds to minutes of release.
Since neural inhibition is likely to be involved in the analysis of spectrally complex sounds, the finding that the CNC contains major inhibitory net-works is of central importance to auditory brain-stem implant research. The glycine concentration in the CNC is among the highest in the brain (19). Major inhibitory pathways are responsible for the fact that electrophysiological responses to acoustic stimuli are heavily complicated by inhibitory interactions in DCN, but not to the same degree in VCN (21). There are prominent intranuclear projections from VCN to DCN (4), and vice versa (4,22), mostly to tono-topically corresponding areas. These pathways are likely to contribute to the inhibition phenomena seen in the subnuclei (21).
Like other sensory systems, the auditory system goes through sensitive periods during early post-natal development. For these periods, sensory input itself assumes a major function in shaping brain architecture (23). These plastic periods must go along with specific molecular events. Available markers for axonal sprouting, such as GAP-43, suggest that plasticity has ceased in the cochlear nucleus of the adult mammal (24). However, a potential to reactivate plastic events appears to exist in this nucleus. Experimental manipulations may succeed in the activation of regu-latory genes such as c-fos (25), which in turn can increase neurotropin synthesis and synthesis of neurotropin receptors (26) in the adult CNC. At present, however, we know much too little about this potential to utilize it for rendering the brainstem optimally receptive towards auditory implants.
To summarize, neurobiological observations on subhuman mam-malian species appear to recommend an artificial substitute to the auditory nerve to be placed over, or into, the ventral rather than the dorsal cochlear nucleus for several reasons. Compared to DCN, VCN is larger, receives more primary auditory fibers, is dominated by this input, involves activation of fewer local inhibitory elements, and projects more heavily along the 'spectral line' to the inferior colli-culus. Moreover, stimulation of VCN would also cause tonotopically corres-ponding excitation of neurons in DCN. The data seem also to indicate that a synchronous stimulation of VCN and DCN could lead to a significant mutual blockade of both CNC subdivisions by way of intranuclear inhibitory connections. The primary-like response character-istics of bushy cells would appear to suggest that experiences made with coding strategies for the cochlear implant may be utilized for brain stem implants when placed over VCN.
(1) Kaltenbach JA, Lazor J (1991) Hear. Res. 51: 149-160.
(2) Osen KK (1969) J. Comp. Neurol. 136: 453-484.
(3) Rose JE, Galambos R, Hughes JR (1959) Bull. Johns Hopkins Hosp. 104: 211-251. (3) Rose JE, Galambos R, Hughes JR (1959) Bull. Johns Hopkins Hosp. 104: 211-251.
(4) Snyder RL, Leake PA (1988) J. Comp. Neurol. 278: 209-225; Morest DK, Kane EC (1974) J. Comp. Neurol. 155 251-300. (4) Snyder RL, Leake PA (1988) J. Comp. Neurol. 278: 209-225; Morest DK, Kane EC (1974) J. Comp. Neurol. 155 251-300. (5) Fekete DM, Roullier EM, Liberman MC, Ryugo DK (1984) J. Comp. Neurol. 229: 432-450.
(5) Fekete DM, Roullier EM, Liberman MC, Ryugo DK (1984) J. Comp. Neurol. 229: 432-450.
(6) Lorente de No R (1981) The Primary Acoustic Nuclei. Raven, New York. (6) Lorente de No R (1981) The Primary Acoustic Nuclei. Raven, New York.
(7) Cant NB, Morest DK (1978) Neuroscience 3: 313-326. (7) Cant NB, Morest DK (1978) Neuroscience 3: 313-326.
(8) Koerber KC, Pfeiffer RP, Warr WB, Kiang NYS (1966) Exp. Neurol. 16: 119-130; (8) Koerber KC, Pfeiffer RP, Warr WB, Kiang NYS (1966) Exp. Neurol. 16: 119-130;
(9) Adams JC (1979) J. Comp. Neurol. 183: 519-538. (9) Adams JC (1979) J. Comp. Neurol. 183: 519-538.
(10) Brawer JR, Morest DK, Kane EC (1974) J. Comp. Neurol. 155: 251-300. (10) Brawer JR, Morest DK, Kane EC (1974) J. Comp. Neurol. 155: 251-300.
(11) Brawer JR, Morest DK (1975) J. Comp. Neurol. 160: 491-506. (11) Brawer JR, Morest DK (1975) J. Comp. Neurol. 160: 491-506.
(12) Rhode WS, Oertel D, Smith PH (1983) J. Comp. Neurol. 213: 448-463. (12) Rhode WS, Oertel D, Smith PH (1983) J. Comp. Neurol. 213: 448-463.
(13) Cant NB, Casseday JH (1986) J. Comp. Neurol. 247: 457-476. (13) Cant NB, Casseday JH (1986) J. Comp. Neurol. 247: 457-476. (14) Cant NB (1982) Neurosci. Lett. 32: 241-246.
(14) Cant NB (1982) Neurosci. Lett. 32: 241-246.
(15) Rhode WS, Smith PH, Oertel D (1983) J. Comp. Neurol. 213: 426-447. (15) Rhode WS, Smith PH, Oertel D (1983) J. Comp. Neurol. 213: 426-447.
(16) Adams JC (1983) Neurosci. Lett. 37: 205-208. (16) Adams JC (1983) Neurosci. Lett. 37: 205-208.
(17) Martin MR (1983) In: Drescher DG (ed.) Auditory Biochemistry. CC Thomas, Springfield, pp 184-197.
(18) Ottersen OP, Storm-Mathiesen J (1984) In: Björklund A, Hökfelt T (eds.) Handbook of Chemical Neuroanatomy. Vol. 3. Elsevier, Amsterdam. (18) Ottersen OP, Storm-Mathiesen J (1984) In: Björklund A, Hökfelt T (eds.) Handbook of Chemical Neuroanatomy. Vol. 3. Elsevier, Amsterdam.
(19) Godfrey DA, Carter JA, Berger SJ, Lowry DH, Matschinsky FM (1977) J. Histochem. Cytochem. 25: 417-431; Wenthold RJ, Huie D, Altschuler RA, Reeks KA (1987) Neuroscience 22: 897-912. (19) Godfrey DA, Carter JA, Berger SJ, Lowry DH, Matschinsky FM (1977) J. Histochem. Cytochem. 25: 417-431; Wenthold RJ, Huie D, Altschuler RA, Reeks KA (1987) Neuroscience 22: 897-912.
(20) Glendenning KK, Baker BN (1988) J. Comp. Neurol. 275: 288-308. (20) Glendenning KK, Baker BN (1988) J. Comp. Neurol. 275: 288-308.
(21) Evans EF, Nelson PG (1973) Exp. Brain Res. 17: 402-427; Evans EF, Nelson PG (1973) Exp. Brain Res. 17: 428-442; Evans EF, Zhao W (1993) Prog. Brain Res. 97: 117-126. (21) Evans EF, Nelson PG (1973) Exp. Brain Res. 17: 402-427; Evans EF, Nelson PG (1973) Exp. Brain Res. 17: 428-442; Evans EF, Zhao W (1993) Prog. Brain Res. 97: 117-126.
(22) Wickesberg RE, Oertel D (1988) J. Comp. Neurol. 268: 389-399. (22) Wickesberg RE, Oertel D (1988) J. Comp. Neurol. 268: 389-399.
(23) Coleman J, Blatchley BJ, Williams JE (1982) Dev. Brain Res. 4: 119-123; Clopton BM, Snead CR (1990) In: Coleman JR (ed.) Development of Sensory Systems in Mammals. Wiley, New York, pp 317-338. (23) Coleman J, Blatchley BJ, Williams JE (1982) Dev. Brain Res. 4: 119-123; Clopton BM, Snead CR (1990) In: Coleman JR (ed.) Development of Sensory Systems in Mammals. Wiley, New York, pp 317-338.
(24) Benowitz LI, Apostolides PJ, Perrone-Bizzozero N, Finkelstein SP, Zwiers H (1988) J. Neurosci. 8: 339-352. (24) Benowitz LI, Apostolides PJ, Perrone-Bizzozero N, Finkelstein SP, Zwiers H (1988) J. Neurosci. 8: 339-352.
(25) Peng ZC, Chen S, Fusco M, Vantini G, Bentivoglio M (1993) Brain Res. 632: 57-67. (25) Peng ZC, Chen S, Fusco M, Vantini G, Bentivoglio M (1993) Brain Res. 632: 57-67.
(26) Hengerer B, Lindholm D, Heumann R, Rüther U, Wagner EF, Thoenen H (1990) Proc. Natl. Acad. Sci. USA 87: 3899-3903; Pioro EP, Cuello AC (1990) Neuroscience 34: 89-110 ; Fusco M, Polato P, Vantini G, Cavicchioli L, Bentivoglio M, Leon A (1991) J. Comp. Neurol. 312: 477-491. (26) Hengerer B, Lindholm D, Heumann R, Rüther U, Wagner EF, Thoenen H (1990) Proc. Natl. Acad. Sci. USA 87: 3899-3903; Pioro EP, Cuello AC (1990) Neuroscience 34: 89-110 ; Fusco M, Polato P, Vantini G, Cavicchioli L, Bentivoglio M, Leon A (1991) J. Comp. Neurol. 312: 477-49.
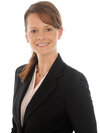
Dr. rer. nat. Nicole Roßkothen-Kuhl
Laborleiterin