SABRE hyperpolarization
Magnetic resonance (MR) is an invaluable tool that has found application in many research fields even though it suffers from an inherent insensitivity. This situation holds true even when the strongest of superconducting magnets are employed, that exceed the earth's magnetic field strength by 100.000 times. Only a miniscule fraction of the nuclear spins present in the corresponding sample contribute positively to the detected MR signal in thermal equilibrium. For the most commonly analyzed spin, 1H (spin 1/2), this fraction, which is in effect the population difference that exists across addressable spin states, amounts to only 3 ppm / T. This situation is far worse for all other stable nuclei because of their weaker interaction with the magnetic field.
Hyperpolarization methods that circumvent this poor thermal distribution of spins have been discussed and employed for decades. Various sources of such spin order have been used which include polarized light (1–4), electron spin (5–8) and parahydrogen (pH2) (9–14). pH2, the spin-singlet nuclear spin isomer of dihydrogen, was suggested for MR signal enhancement in the 1980's (pH2 and synthesis allow dramatically nuclear alignment, PASADENA or pH2 induced polarization, PHIP)(9–11). This method traditionally relies on adding the spin order of one pH2 molecule into a target molecule, a dihydrogen acceptor, by means of (a one-way) catalytic hydrogenation reaction. Many studies have been reported on using this phenomenon as a probe in catalysis and even biomedical in vivo imaging. Some of these results rely on transfer to longer lived 13C hyperpolarization by means of r.f. sequence (13,15) and appropriate experimental equipment has been decribed (16–18). While the associated r.f. sequences are sensitive to B1 (19) and require tedious calibrations (e.g. aided by high-field systems) (20), it has enabled useful pH2-based 13C hyperpolarization to be achieved in biologically relevant agents in aqueous solution14. The detection and quantification of such 13C polarization, in situ, has been reported in (21).
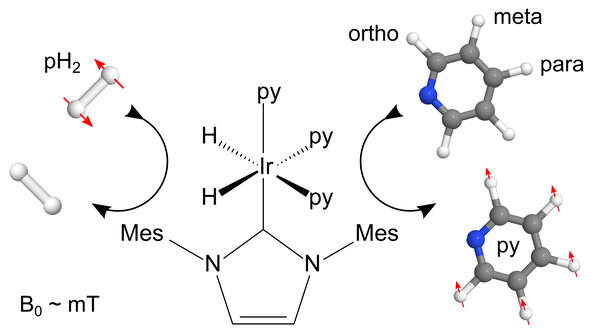
Figure 1: Schematic representation of the SABRE hyperpolarization method using an iridium-based polarization transfer catalyst: the pure but NMR invisible spin order of pH2 is transferred to the pyridine target during their temporary contact which is mediated by the metal complex. Red arrows indicate how spin order equilibration leads to hyperpolarization in the 1H nuclei of pyridine
In 2009, it was demonstrated that the use of pH2 could be extended through what is the catalytic and reversible exchange of the spin order into a target (SABRE)(22,23) In contrast to the traditional hydrogenative pH2-based method, this reversible exchange leaves the hyperpolarized agent chemically unchanged (Fig. 1). It is also not limited to one pH2 molecule per target and hence multiple pump steps lead to impressive levels of hyperpolarization. This effect has also been shown to transfer polarization to 13C, 19F, 31P and 15N nuclei. This method holds great potential for biomedical research, as the proof-of-principle for low-field hyperpolarization of amino acids24 was described using Crabtree's catalyst. The actual hyperpolarization yield in low field, though, was not quantified.
References
1. Bhaskar, N. D.; Happer, W.; McClelland, T. Phys. Rev. Lett. 1982, 49, 25–28.
2. Ebert, M.; Grossmann, T.; Heil, W.; Surkau, R.; Leduc, M.; Bachert, P.; Knopp, M. V.; Schad, L. R.; Thelen, M. Lancet 1996, 347, 1297–1299.
3. Albert, M. S.; Cates, G. D.; Driehuys, B.; Happer, W.; Saam, B.; Springer, C. S.; Wishnia, A. , Published online: 21 July 1994; | doi:10.1038/370199a0 1994, 370, 199–201.
4. Schröder, L.; Lowery, T. J.; Hilty, C.; Wemmer, D. E.; Pines, A. Science 2006, 314, 446–449.
5. Hausser, K. H.; Stehlik, D. Advances in Magnetic Resonance 1968, 3.
6. Abragam, A.; Goldman, M. Rep. Prog. Phys. 1978, 41, 395–467.
7. Ardenkjaer-Larsen, J. H.; Fridlund, B.; Gram, A.; Hansson, G.; Hansson, L.; Lerche, M. H.; Servin, R.; Thaning, M.; Golman, K. Proc. Natl. Acad. Sci. U. S. A. 2003, 100, 10158–10163.
8. Batel, M.; Krajewski, M.; Weiss, K.; Däpp, A.; Hunkeler, A.; Pruessmann, K.; Boesinger, P.; Meiner, B. H.; Kozerke, S.; Ernst, M. Journal Of Magnetic Resonance 2012, 214, 166–174.
9. Bowers, C. R.; Weitekamp, D. P. Phys. Rev. Lett. 1986, 57, 2645–2648.
10. Bowers, C. R.; Weitekamp, D. P. J Am Chem Soc 1987, 109, 5541–5542.
11. Eisenschmid, T. C.; Kirss, R. U.; Deutsch, P. P.; Hommeltoft, S. I.; Eisenberg, R.; Bargon, J.; Lawler, R. G.; Balch, A. L. J. Am. Chem. Soc. 1987, 109, 8089–8091.
12. Haake, M.; Natterer, J.; Bargon, J. Journal of Americal Chemical Society 1996, 118, 8688–8691.
13. Goldman, M.; Johannesson, H. C. R. Physique 2005, 6, 575–581.
14. Chekmenev, E. Y.; Hövener, J.-B.; Norton, V. A.; Harris, K. C.; Batchelder, L. S.; Bhattacharya, P.; Ross, B. D.; Weitekamp, D. P. Journal of the American Chemical Society 2008, 130, 4212–4213.
15. Goldman, M.; Johannesson, H.; Axelsson, O.; Karlsson, M. C. R. Chimie 2006, 9, 357–363.
16. Goldman, M.; Johannesson, H.; Axelsson, O.; Karlsson, M. Magn. Reson. Imaging 2005, 23, 153–157.
17. Hövener, J.-B.; Chekmenev, E. Y.; Norton, V. A.; Weitekamp, R.; Harris, K. C.; Perman, W. H.; Robertson, L.; Weitekamp, D. P.; Ross, B. D.; Bhattacharya, P. Magnetic Resonance Materials in Biology, Physics, and Medicine 2009, 22, 111–121.
18. Kadlecek, S.; Vahdat, V.; Nakayama, T.; Ng, D.; Emami, K.; Rizi, R. NMR Biomed 2011, 24, 933–942.
19. Bär, S.; Lange, T.; Leibfritz, D.; Hennig, J.; Elverfeldt, D. V.; Hövener, J.-B. J. Magn. Reson. in press, DOI 10.1016/j.jmr.2012.08.016.
20. Hövener, J.-B.; Chekmenev, E. Y.; Norton, V. A.; Harris, K. C.; Perman, W. H.; Weitekamp, D. P.; Ross, B. D.; Bhattacharya, P. Magnetic Resonance Materials in Biology, Physics, and Medicine 2009, 22, 123–134.
Dr. Andreas B. Schmidt
Head of Hyperpolarization
Tel.: +49 761 270-93880
E-Mail: andreas.schmidt@uniklinik-freiburg.de
University Medical Center Freiburg
Dept. of Radiology · Medical Physics
Killianstr. 5a
79106 Freiburg